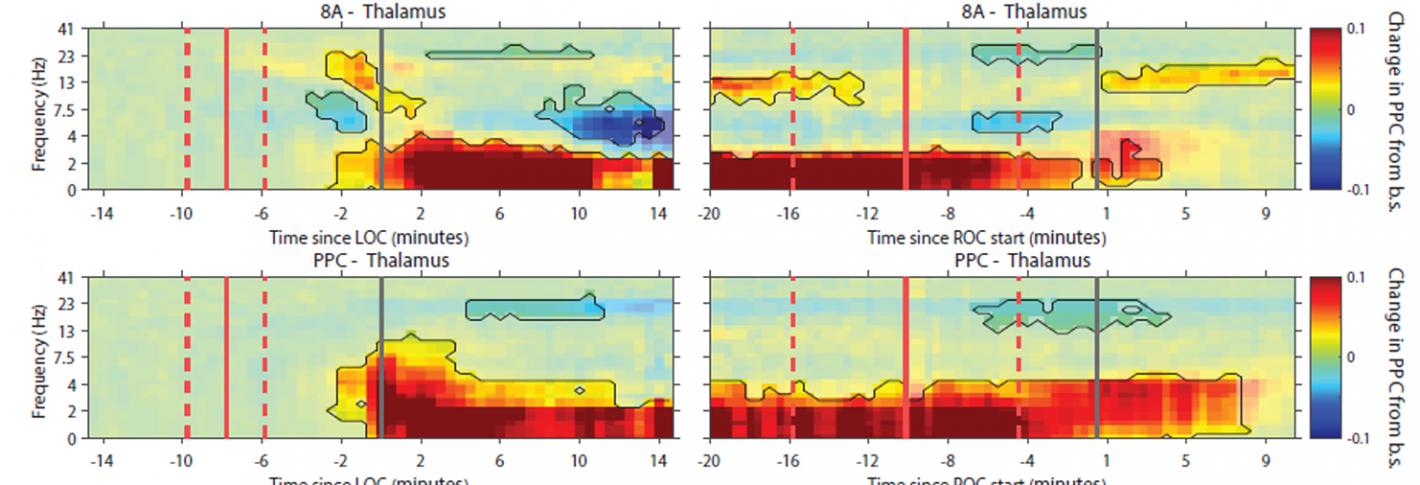
Though it has not been traditionally studied with a neuroscience foundation, general anesthesia works in the brain and central nervous system to induce four states: unconsciousness, immobility, amnesia and suppression of the body’s damage sensing response, or “nociception,” which would be sensed as pain during consciousness. Dedicated to establishing a rigorous neuroscience basis for the anesthesiology field, Emery N. Brown, Edward Hood Taplin Professor of Medical Engineering and Computational Neuroscience at MIT, has helped to lead numerous discoveries about how anesthetic drugs affect the brain, both before and after joining The Picower Institute in 2015.
Notably, Brown, who is also a statistician and practicing anesthesiologist at Massachusetts General Hospital, has found important distinctions in the effects and underlying neural mechanisms among commonly used anesthetic drugs (for instance between propofol and ketamine). Their distinct effects on brain circuits and chemistry, he and his collaborators have shown, lead to “signatures” that are readily evident in the real-time electroencephalogram (EEG) measurements that anesthesiologists can make and monitor in the operating room. Doctors can use these readings to more precisely and directly monitor unconsciousness and drug dosing to minimize side effects. Moreover, neuroscientists can use these findings to better understand how the changes in brain rhythms, as evidenced in the EEG measurements, alter consciousness, memory, motor function and nociception.
Ketamine
In 2016, Brown and collaborators looked at EEG recordings made from 12 surgery patients who received ketamine to induce general anesthesia. Their statistical analysis revealed that ketamine produced an EEG pattern that consisted of alternating slow-delta (0.1–4 Hz) and gamma (∼27–40 Hz) rhythms along with increased theta rhythms (∼4–8 Hz) and decreased alpha/beta ones (∼10–24 Hz), compared to the conscious state. Then in 2021 Brown and Picower Institute colleague Earl K. Miller followed up with an analysis of nine human volunteers and two animal subjects. They produced a “hidden Markov model” that defines the distinct, characteristic states of brain activity that occur during ketamine-induced anesthesia, including how long each lasts. The model also tracks patterns of how the states switch from one to the next, therefore providing anesthesiologists, neuroscientists, and data scientists alike with a principled guide to how ketamine anesthesia affects the brain and what patients will experience.
Dexmedetomidine
In 2016 Brown and co-authors also performed a detailed signal processing analysis iof the EEG readings produced in the brains of eight volunteers who were administered dexmedetomidine. They showed that the anesthetic was associated with increased slow-delta oscillations and decreased beta across the entire scalp, increased theta oscillations in occipital regions, increased spindle oscillations in frontal regions. Overall, though general anesthesia is very different from natural sleep, in this case the readings suggested that induction of unconsciousness with this medication closely approximated the onset of sleep. In another study the following year, Brown and colleagues analyzed brain network activity in a larger group of volunteers under dexmedetomidine general anesthesia. The team discovered that the drug reduced network efficiency and connectivity strength. That meant that the drug was associated with a significant drop in the capacity for efficient information transmission at both the local and global levels, which likely contributes to how it induces unconsciousness.
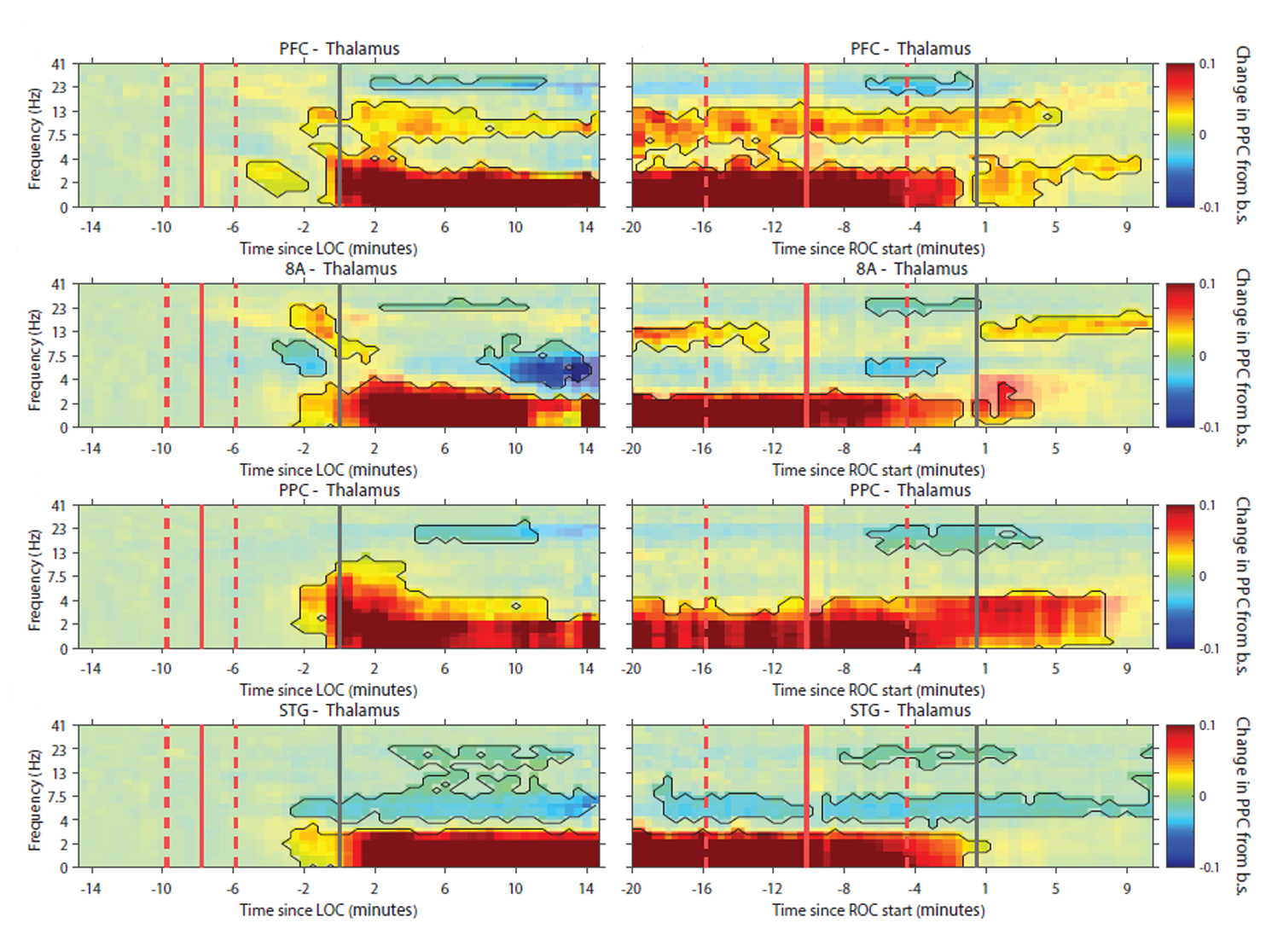
Propofol
Brown and his colleagues have examined the brain effects of the common anesthetic drug propofol in several studies. In 2016, for instance, by making measurements in two different regions of the cortex in animals during induction of propofol anesthesia, they showed that beta rhythm coherence between the regions become disrupted before loss of consciousness followed by peaks of gamma rhythms around loss of consciousness at different times in the two regions. After loss of consciousness, much slower rhythms took over in one region and then the next. The findings showed that when propofol induces unconsciousness, there are spatially and temporally distinct effects on brain rhythms. The next year Brown and colleagues including Picower Institute colleague Matt Wilson found in rats that normal conscious activity between the thalamus and medial prefrontal cortex become disrupted by propofol. Alpha oscillations (10–15 Hz) become synchronized and, at deep levels of unconsciousness, coherent delta oscillations (1–5 Hz) also developed.
In 2021, Brown worked with Picower Institute colleague Earl K. Miller, to measure both rhythms and individual neural spikes across four cortical regions and the thalamus in animals undergoing propofol anesthesia. While conscious function depends on coordinated brain communication between the thalamus and the cortex in a variety of frequency bands, they discovered that propofol seems to bring coordination among the thalamus and cortical regions down to frequencies around just 1 Hz.
Sevoflurane
Before a 2019 study by Brown and colleagues, some scientists hypothesized that sevoflurane induced general anesthesia in part by producing coherent frontal alpha rhythms. Because that had not been proven, Brown’s team sought to investigate by studying the effects on 12 subjects who received either sevoflurane alone or the common pairing of sevoflurane and ketamine (ketamine, as noted above, reduces alpha rhythms). The results indicated that coherent alpha may not be necessary for sevoflurane to maintain general anesthesia. Instead of anesthetic-induced unconsciousness tracking with the power of a particular wave frequency, they found, anesthetic brain states under sevoflurane emerged from impaired information processing caused by how the delta rhythms and higher frequencies rhythms coupled together. In other words, the way that delta waves restricted the timing of higher-frequency rhythms is what mattered.
Nitrous Oxide
A method of facilitating recovery from sevoflurane general anesthesia is to transition the patient to nitrous oxide. In 2016, Brown and colleagues revealed the effects of this transition on brain rhythms. An apparent signature of alpha (8–12 Hz) rhythms associated with sevoflurane (see above) dissipated within about six minutes in favor of highly coherent, large-amplitude slow-delta (0.1–4 Hz) oscillations that persisted for another few minutes. Based on the action of the drug in the brain the team postulated that the slow-delta oscillations resulted from nitrous oxide blockading excitatory input from brainstem regions to cognition-producing regions including the thalamus and cortex.