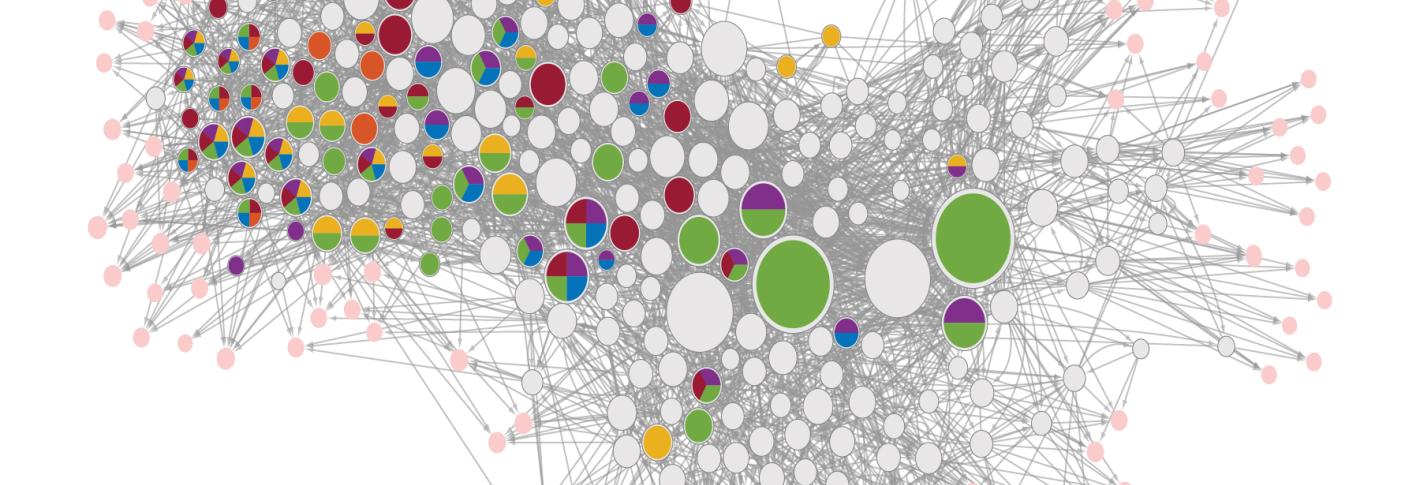
Your brain is like a biological pharmacy. To regulate your internal states, such as arousal and mood, and your responses to the world, such as your sense of reward when you do something right or surprise when the results are unexpected, it dispenses doses of natural “drugs” called neuromodulators. Under healthy circumstances, these chemicals steer the activity of neurons to produce and sustain relatively long-lasting feelings and behaviors and then switches them to a different mode of activity when changing conditions warrant.
Trained by hundreds of millions of years of evolution, nervous systems do this with ease and precision. They synthesize and “prescribe” neuromodulators—serotonin, dopamine, endocannabinoids, norepinephrine, etc.—whenever and where ever they are needed, molecularly tweaking how neurons respond to the electrochemical signals they receive within the circuits they inhabit. In this way, neuromodulators can change the output of a circuit involved in a state or behavior.
With appreciation of the extraordinary influence of neuromodulators, humans have attempted to follow nature’s example. We create or find drugs, such as Prozac, Ritalin, marijuana, psilocybin or MDMA, that manipulate neuromodulatory mechanisms in a quest to feel better.
But the imperfect efficacy of many pharmaceutical treatments and their significant risk of side effects lays bare a paradox: Like drugs, neuromodulators wash over millions of cells but they nevertheless achieve their broad-based effects quite precisely, finding the right receptors, on the right neurons at the right times in the right places even when multiple neuromodulators are all working in parallel in a somehow perfectly balanced soup. With incomplete knowledge of how the brain does this in health—and how it falters in disease—neuroscientists, drug designers, and psychiatrists haven’t replicated the ability nature has evolved to affect neural circuits both broadly and precisely.
That is a main reason why improving fundamental understanding of how neuromodulation works is a priority among many researchers in The Picower Institute for Learning and Memory. Looking across different model organisms, different neuromodulators, and different behaviors and outcomes, they have published many recent studies shedding light on the mechanisms by which neuromodulators exert their effects. By adding to the knowledge of the field, they can help science catch up to nature, both in understanding how nervous systems work and how to better treat them. The field has made progress, of course, but much more is needed.
“We have a basic understanding of many modulatory systems, for example we know which neurons release serotonin and understand basic pharmacology of many serotonin receptors. But these neuromodulators can impact literally millions of neurons all at the same time,” said Associate Professor and HHMI Investigator Steve Flavell. “There’s not a strong understanding of the way that this parallel action on a million different units at once changes the way that animals are thinking, perceiving, feeling and acting. It’s just really poorly understood.”
Many psychiatric drugs, such as Prozac, seek to increase or decrease overall levels of neuromodulators, for instance by preventing cells from reabsorbing them. Sometimes this works, but in other cases it fails. The approach may be too coarse. More precise therapies, and ones with fewer side effects, will require figuring out the exact underlying mechanisms governing when neuromodulators are released, where they go, and how they affect their neural targets individually and en masse.
“We still don’t understand how Prozac has such a specific effect in some cases and not in others, and we also don’t understand why serotonin or norepinephrine reuptake inhibitors work,” said Newton Professor Mriganka Sur. “It’s lucky that we do get some efficacy. Based on function and the mechanism, people will one day build very specific molecules that affect very specific brain circuits.”
Assistant Professor Brady Weissbourd agrees: “We’re increasingly appreciating the complexity and specificity of these neuromodulatory systems and precisely when and where a modulator is released. The spatiotemporal dynamics of all that are super important. A need for more targeted manipulations is going to be key.”
Examples of new findings about neuromodulatory mechanisms abound in Picower labs. This year in Nature, for instance, Picower Professor Li-Huei Tsai discovered that using light and sound to stimulate a particular brain rhythm in mice increases the release of the neuromodulatory neuropeptide VIP, which in turn compels astrocyte cells and the brain’s vasculature to increase clearance of toxic waste proteins whose buildup is associated with Alzheimer’s disease. Her lab is now investigating further roles that neuropeptides may have in the potential therapy.
“We still don’t understand how Prozac has such a specific effect in some cases and not in others, and we also don’t understand why serotonin or norepinephrine reuptake inhibitors work,” said Newton Professor Mriganka Sur. “It’s lucky that we do get some efficacy. Based on function and the mechanism, people will one day build very specific molecules that affect very specific brain circuits.”
For another example, in 2021 Associate Professor Gloria Choi found in Nature that the neuromodulator TRH was an essential component in switching neural circuit activity in male mice, such that when they smelled that females were sick, they’d forgo their typical mating instinct—a form of social distancing mediated by a neuromodulator. Moreover, Choi has published several other studies showing that immune system molecules called cytokines may have neuromodulatory properties, for instance by affecting social behavior when they act on cortical neurons. She has also seen initial evidence that cytokines might affect mood.
Serotonin system-wide
Flavell has been studying the simple C. elegans nematode to decipher a natural model of how serotonin works systemically across a whole animal. The transparent, microscopic worm has only 302 neurons (compared to tens of billions in a human) and has six different serotonin receptors (compared to 14 in people), but that simplicity is an asset, not a liability. The worm’s neural circuits have been completely mapped out and all its genes can be manipulated. All its behaviors can be scrutinized and all its underlying neural activity can be measured. The tractability of C. elegans enabled Flavell’s lab to perform what Weissbourd (who studied serotonin in mice as a graduate student) called a “dream” experiment: Flavell’s team genetically knocked out every possible combination of the worm’s six serotonin receptors so that he could see, brainwide, how that affected a particular serotonin-dependent food seeking behavior. Then the team also fluorescently tagged each receptor to map which neurons had which receptors. And finally, they watched the activity of each neuron amid instances of serotonin release to see how their combination of receptors related to their activity.
The study enabled Flavell to learn the distinct functions of each receptor, alone and in combination. The implications of the study for drug developers was clear: Given that many neurons may express different combinations of serotonin receptors, the effects of targeting one serotonin receptor or another could vary depending on how other receptors or the cell types that express them are functioning. In particular, the study highlighted how distinct serotonin receptors act in concert to change the activity states of neural circuits.
Flavell plans new experiments to build on these results. First he wants to drill down to see where each neuron expresses its various serotonin receptors and determine the logic of why they are where they are. To expose the “hidden variables” of where serotonin goes when it is released, how quickly it gets there and how long its stays, he plans to use optical labels of serotonin developed by Yulong Li at Peking University. And Flavell also hopes to work with the C. elegans model to tackle another problem that is very difficult to deal with in more complex organisms: how different neuromodulators work in combination.
“Individual cells have receptors for many of these neuromodulators and they’re all acting together,” Flavell said. “Any satisfactory understanding of how these systems work is inherently going to have to address the combinatorial nature of these neuromodulators.”
Modulating memory
While Flavell is studying neuromodulators systematically across the worm, Sur and Assistant Professor Linlin Fan have each been investigating specific mechanisms in mice that demonstrate neuromodulators’ relevance to learning and memory.
Earlier this year, Fan co-led a study in Science that identified a role for endocannabinoids in a process by which cells in the brain’s hippocampus encode memories of specific locations. Using innovative optical techniques to stimulate and record electrical activity in the cells involved, Fan’s team also used Li’s optical neuromodulator labels to track the flow of endocannabinoids in living mice. The study provided suggestive evidence that when a “place cell” is being stimulated to remember a location, it uses a burst of endocannabinoids to quiet down inhibitory signals that would otherwise come from another neuron. To prove it, Fan plans new experiments in which she’ll knock out the endocannabinoid receptors on the inhibitory cells and see what difference that makes to the electrical activity of the place cells. If the hypothesis is true, then the inhibitory neurons that lack endocannabinoid receptors, should persist in hindering the place cells’ efforts to tune to the location they are learning.
The significance of understanding how endocannabinoids help to implement learning and memory goes beyond the goal of understanding how the brain achieves a refined memory of where it’s been. Endocannabinoid release increases amid epileptic seizures, so the study could shed light on how neural circuits might become perturbed when that happens. And because marijuana supplies the brain with external endocannabinoids, the research could help inform questions about how the drug might affect natural memory formation processes.
Sur’s studies, meanwhile, have concerned how a different neuromodulator, norepinephrine, affects learning in different areas of the brain.
Traditionally dopamine has been associated with learning from reward and norepinephrine has been associated with arousal. But the two molecules are similar and even have the same chemical precursor, Sur said. Therefore Sur decided to investigate whether norepinephrine might have a role in learning from reward (and punishment), like its cousin dopamine.
This line of research in Sur’s lab reached a major milestone in 2022 with their paper in Nature, identifying specific mechanisms by which norepinephrine indeed influences learning. In the study the scientists rewarded mice with a refreshing sip of water if they pushed a lever when they heard a high-pitched tone, but gave them a little irritating puff of air if they pushed the lever when they heard a low-pitched tone. During the study the researchers varied each tone’s loudness, thereby varying the certainty the mice had about whether they heard a tone and which one. Meanwhile, the researchers tracked and manipulated neural activity in the locus coeruleus, where norepinephrine is made, and monitored activity in other brain regions.
The study yielded several insights into how norepinephrine mattered to the task. One was that after the mouse pushed the lever (or didn’t) in response to tone, norepinephrine reinforced the result. If the outcome was expected, there’d be just a small burst of the neuromodulator, but if the outcome was unexpected (for instance getting a puff of air instead of a reward), then there’d be a massive norepinephrine release in specific brain regions. After experiencing this signal of surprise mice would alter their behavior on the task, immediately factoring in what it learned from the surprising result.
The findings evoked another connection between norepinephrine and learning, Sur said. The neuromodulator is also associated with “single shot” learning, in which all that’s required to indelibly remember an especially shocking stimulus is a single exposure. An unfortunate example is post-traumatic stress disorder.
“Norepinephrine marks surprising events,” Sur said. “We know it’s a marker of single shot events, good or bad and the bad ones need to be treated. We think that knowing the norepinephrine source, and the target that underlies this memory, would be a very focused target for treating such disorders.”
Evolving understanding
To Sur, one of the best testaments to the importance of neuromodulatory chemistry is its deep evolutionary roots.
“It exists in many of the humblest organisms, which leads us to ask: Why? What do they do?,” Sur said. “They must have very profound functions.”
One of those humble and evolutionarily distant organisms is Flavell’s worms, which not only employ serotonin but also dopamine and other neuromodulators found in humans. An even more distant relative is the Clytia hemisphaerica jellyfish that Weissbourd studies. The branch of the evolutionary tree that the jellyfish descended along split off from the one humans emerged from right after the first nervous systems evolved.
“By comparing between jellyfish and other model organisms we can ask questions about what are the sort of deeply conserved principles of how our nervous systems work,” he said.
While jellyfish don’t make serotonin or dopamine, Weissbourd has found that they employ about two dozen neuropeptides to send signals around their neural networks (even before there were neurons, per se, cells were using such peptides to communicate with one another). Weissbourd is systematically studying how each peptide affects the jellyfish’s behaviors, both by knocking out the cells that make them, and seeing how behavior differs, and by synthesizing the peptides and seeing what happens when they are administered. If indeed at least some of the peptides are acting as neuromodulators—for instance by broadly yet precisely influencing circuit activity to represent and switch among internal states—then that would help confirm that even in this most distant of nervous systems, neuromodulation is still at work.
“I think there is a very ancient underpinning of how circuits can be modulated and flexible,” Weissbourd speculated. “I think that dates back to the origins of nervous systems and may be conserved across all nervous systems.”
This suggests that when we seek to manipulate our neuromodulatory systems with medicines or other drugs, we’re tapping into a system that nature has been refining since before the dinosaurs. Only through research can scientists and clinicians hope to catch up.